Yinan Chen, Song Wu, [...], and Peter Svensson
Abstract
Objective
To compare the effects of training of jaw and finger movements with and without visual feedback on precision and accuracy.
Method
Twenty healthy participants (10 men and 10 women; mean age 24.6 ± 0.8 years) performed two tasks: a jaw open-close movement and a finger lifting task with and without visual feedback before and after 3-day training. Individually determined target positions for the jaw corresponded to 50% of the maximal jaw opening position, and a fixed target position of 20 mm was set for the finger. Movements were repeated 10 times each. The variability in the amplitude of the movements was expressed as percentage in relation to the target position (Daccu—accuracy) and as coefficient of variation (CVprec—precision).
Result
D accu and CVprec were significantly influenced by visual feedback (P = 0.001 and P < 0.001, respectively) and reduced after training jaw and finger movements (P < 0.001). Daccu (P = 0.004) and CVprec (P = 0.019) were significantly different between jaw and finger movements. The relative changes in Daccu (P = 0.017) and CVprec (P = 0.027) were different from pretraining to posttraining between jaw and finger movements.
Conclusion
The accuracy and precision of standardized jaw and finger movements are dependent on visual feedback and appears to improve more by training in the trigeminal system possibly reflecting significant neuroplasticity in motor control mechanisms.
1. Introduction
Neuroplasticity is one of the most prominent features of the central nervous system, which plays a role in function to store information in memory associated with learning [1]. In healthy humans, gain in overall motor performance, reflective of motor learning, has been associated with neuroplasticity of the motor cortical territories corresponding to the trained muscles and can occur after short-term (hours to days) motor training regimes [2–4].
Previous studies have shown that the neuroplasticity of corticomotor pathways can be established after a series of repetitive motor tasks such as tooth clenching [5, 6], tongue movements, hand movements [7, 8], and leg movements [9]. It has been suggested that motor training results in improvements in the precision of the task performance with increased representation of the trained muscle in the motor cortex [3, 8].
Repetitive jaw open-close movements are one of the basic components of physiological jaw motor actions during mastication. Open-close jaw movements must achieve a predetermined requirement with high precision in mastication, a complex biomechanical process, involving positioning food between the teeth, holding, breaking it down into pieces to prepare it for swallowing [10]. The essential rhythm of jaw movements is determined by a group of neurons in the brain stem termed the masticatory CPG (central pattern generator) [10–12]. The CPG is initiated by the inputs from higher centers (motor cortex) and is influenced by somatosensory information from the orofacial mechanoreceptors [10, 11]. Therefore, it is suggested that the somatosensory cortex and the motor cortex are important in the mastication process, e.g., chewing and swallowing. The primary motor cortex is involved in the initiation, control, and execution of the jaw motor functions and also plays an important role in the acquisition of new motor skills [13].
Recent research on oral motor performance has demonstrated the ability to increase performance and skill acquisition during different orofacial motor tasks, for example, manipulation and splitting spherical pieces of candy into two almost equal halves following a short-term training (about 30 min) [14]. The results of that study showed that the participants were able to successfully perform the task with increased levels of precision following a short-term training. Another recent study by Kumar et al. investigated the dynamic changes in accuracy and precision during a simple oral and digital motor task involving a controlled and a ballistic force. That study demonstrated differences between accuracy and precision of training-related dynamic modulations of forces due to repeated performance; i.e., it may be important to consider both accuracy and precision in the evaluation of neuroplastic changes in motor skills [15].
There may also be differences in motor control programs between hand and jaw muscles reflecting anatomical and functional differences between the trigeminal and spinal system. Iida et al. used functional magnetic resonance imaging (fMRI) to provide more information on the central processing mechanisms underlying awake bruxism and directly compared cerebral activity between bilateral light fist clenching and light tooth clenching [16]. Interestingly, the results showed that a less complex pattern of cerebral activity was induced by the hand motor task compared to the performance of the tooth clenching [16]. Another recent study recorded electromyography (EMG) and force in repetitive finger pinch and tooth clenching tasks and demonstrated that motor learning for the jaw motor system is more likely to occur than that for the hand motor system [17]. It seems that information on neuroplastic changes evoked by motor training cannot directly be extrapolated from the spinal system to the trigeminal system.
In previous studies, the behavioral consequences of motor learning have been characterized by two descriptive statistics, a mean and a variance, the former meaning accuracy, also referred to as “constant error” and the latter meaning precision, sensitivity, discrimination threshold, or “temporal variance” [18, 19]. However, no study has so far compared both the accuracy and precision of jaw and finger movements in terms of training-induced neuroplastic effects and motor performance.
The hypothesis of the present study was that the accuracy and precision of jaw and finger movements would increase after short-term training paradigms with no differences between the trigeminally and spinally innervated muscles.
2. Materials and Methods
2.1. Participants
Twenty young adults (ten men and ten women; mean age 24.6 ± 0.8 years) were recruited among the students at Nanjing Medical University. All participants were healthy individuals with no signs or symptoms of pain in the head, face, and the right index finger and absence of any jaw movement restrictions that involved the masticatory musculature, the temporomandibular joint, and associated structures. The exclusion criteria included past trauma to the jaw and right arm or hand, a history of systemic illnesses to joints or related muscles, ongoing dental treatment, mental disorders, peripheral neuropathy, presence of any acute or chronic orofacial pain conditions or taking medications for pain, or neurological diseases. The study was conducted in accordance with the guidelines set forth in the Declaration of Helsinki II, and informed consent was obtained from all participants prior to participation. The study was approved by the Nanjing Medical University Research Ethics Committee (No. PJ2015-040-001).
2.2. Recording Equipment
A kinesiograph (K7/CMS; Myotronics, Inc., USA) was used to record jaw and finger movements with an accuracy of 0.1 mm and a sampling frequency of 50 Hz. An array attached to a headset containing 8 magnetic sensors tracked the motion of a magnet (CMS Magnet; Myotronics, Inc., USA) attached to the lower incisors in the midsagittal plan or the nail of the right index finger (Figure 1). The magnet was positioned based on anatomical guidelines and reference points with a test-retest variability < 1 mm. The movement data was sampled, stored, and analyzed by a commercial software program (K7 Program, Myotronics, Inc., USA).
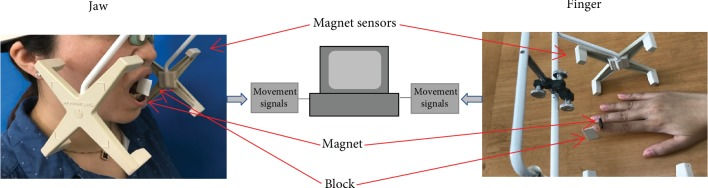
Illustration of the movement recording equipment applied to the jaw and finger. Magnet attached to lower incisors in the midline or to the nail of the right index finger. Magnet sensors (4 × 2) in the headset recorded the vertical dimension of ...
2.3. Recording Procedure
All participants were examined twice in two separate sessions in this study (Figure 2). In each session, participants were asked to perform 10 repeated jaw movements and finger movements to a target position during two trials: trial 1 with visual feedback and trial 2 without visual feedback. In trial 1, all participants kept their eyes open to control their finger movements and they were given a mirror to control their jaw movements. In trial 2, the participants received no visual feedback from the jaw and finger movements as they were asked to keep their eyes closed. The 10 repeated open-close jaw movements and finger lifting movements were considered one trial, respectively. In session 1, all movements were without any prior motor training. In session 2, all participants had repeated the same series of movements for 3 days: three times of motor training for the jaw and finger each day. The pace of the movement was controlled by a metronome set to 10 beats/min (auditory cue) to maintain a similar speed in each trial. All recordings were performed by the same examiner.
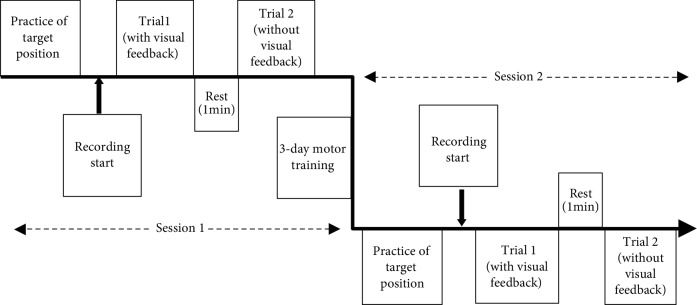
Schematic diagram of the experimental design: session 1—pretraining, session 2—post-training, trial 1—movements with visual feedback, and trial 2—movements without visual feedback.
2.4. Jaw Movements
The participants were seated in a chair in a comfortable upright position with a straight back. The jaw tracking device was mounted on the participants in accordance with previously published papers [20].
At the beginning of the test, participants were instructed to open their jaw as wide as possible without feeling pain starting from the intercuspal position with teeth in light contact for three times to obtain the mean value of the maximal jaw opening. A simple ruler was used to determine the interincisal distance and the horizontal overbite. Then the task was to perform 10 repeated open-close movements to an individually adjusted target position corresponding to 50% of the maximal jaw opening position adjusted for the individual differences in vertical overbite. A plastic block (block 1) was cut for each participant to reproduce the 50% of the maximal jaw opening position as the target position (Figure 1). Prior to each trial, the participants were instructed with the aid of block 1 to open their jaw to reach the target position. Then the open-close jaw movement was performed 10 times without the help of block 1.
2.5. Finger Movements
The participants were comfortably seated in a chair and placed their right hand and arm on the table with the palm of the hand downwards without pressure or efforts. The magnet (CMS Magnet; Myotronics, Inc., USA) was attached to the nail of the right index finger with the hand adjusted to the center of the headset of kinesiograph device (Figure 1). The participants were instructed to lift only the index finger from the resting position on the table in a vertical direction to an amplitude corresponding to 20 mm defined as the target position at the beginning of the test. Another plastic block (block 2) was cut to reproduce the target position for each participant. Prior to each trial, participants were instructed to lift the finger to the target position with the help of block 2 and then the finger movements were performed 10 times without the aid of block 2. We connected the magnet in a straight line and made a vertical line at the midpoint of the line. In the experiment, we placed the midpoint in the center of the nail and overlapped the long axis of the index finger and the vertical line completely in order to minimize variation and to standardize recording conditions.
An additional control experiment was performed in a separate session in eleven other healthy individuals (3 men and 8 women, mean age: 26.5 ± 1.9 years) to determine accuracy and precision at different ranges of amplitudes of the finger lifting task. The amplitude was set at 10, 20, 40, and 60 mm to cover the 50% of the maximal index finger lift capacity. A total of 10 repetitions at the same speed as described above and with the exact same methodology were used to calculate the accuracy and precision with and without visual feedback, but without any subsequent motor training task.
2.6. Motor Training Tasks
A 3-day motor training task at home was initiated following session 1 (Figure 2) for twenty participants. The participants were asked to perform open-close jaw movements and finger lifting movements with the use of the customized plastic blocks (block 1 and block 2) to practice the target position with a metronome to maintain a constant speed. The exercise program for each day was to repeat each set of exercises for 10 times with the help of the plastic blocks with an interval of 2 minutes. Each participant was given a form with timetables to fill in with an “X” when the exercise was done for each day. After 3 days, the same jaw opening-closing and finger lifting movements were repeated two times (with and without visual feedback) in session 2 and assessed by the same examiner (Figure 2).
2.7. Statistical Analysis
The sample size was calculated a priori based on the detection of a minimum relevant difference of 25% at an α level of 0.05 and 80% power (i.e., the risk of a type I and type II error was 5% and 20%, respectively). A total of 20 participants were recruited in each group.
For each participant, the peak values of jaw and finger movements from each trial in the two sessions were determined by the K7 Program (Myotronics, Inc., USA).
The accuracy of the motor behaviors (Daccu) was quantified by the ratio of the absolute error (μ) to the target value (X). It is expressed here as a percentage:

The coefficient of variation (CVprec) was used to quantify the precision of the motor behaviors. The CV is the ratio of the standard deviation (σ) to their mean value (). It is expressed here as a percentage:

The relative changes of Daccu and CVprec in the two trials (with-without visual feedback) from session 1 to session 2 (before-after) were used to compare directly motor performance for jaw and finger movements.
Descriptive statistics were used to summarize the data. The necessary logarithmic transformation was performed when the data was not normally distributed. The mean and SDs of Daccu, CVprec, and relative changes for jaw and finger movements in two trials (with visual feedback/without visual feedback) during session 1 and session 2 (before/after training) were calculated. A three-way analysis of variance (ANOVA) was used to analyze different outcome parameters with the following factors: session (2 levels: before and after training), site (2 levels: jaw and finger), and trial (2 levels: with and without visual feedback). A one-way ANOVA was used to evaluated differences for different amplitudes of the finger lifting task (4 levels: 10, 20, 40, and 60 mm). Post hoc tests were performed with an LSD Honest Significant Difference test with corrections for multiple comparisons. A value of P < 0.05 was considered statistically significant.
3. Results
The mean and SDs of Daccu, CVprec, and absolute changes (before-after) are shown in Table 1 and Figure 3. The mean and SDs of Daccu and CVprec in 4 different amplitudes (10, 20, 40, and 60 mm) in two trials (with/without visual feedback) are shown in Table 2. All individual values for accuracy and precision are shown in Figure 4 and indicate improved performance; i.e., the individual target position values converge towards 100% for both jaw and finger movements, and Daccu and CVprec decrease after the training tasks. The Daccu and CVprec of jaw movement were 4.4 ± 3.2/6.8 ± 2.2 in trial 1 (with visual feedback) and 9.6 ± 9.2/9.2 ± 3.4 in trial 2 (without visual feedback) in session 1 (pretraining). In session 2 (posttraining), the Daccu and CVprec were 1.2 ± 1.1/3.2 ± 0.8 in trial 1 and 3.1 ± 2.7/4.3 ± 1.1 in trial 2. The relative changes (before-after) were 65.6 ± 28.9/51.2 ± 12.5 in trial 1 and 65.1 ± 24.5/48.0 ± 22.1 in trial 2. Daccu of jaw and finger are significantly different at baseline (two types of movement pretraining with visual feedback). However, no significant differences of CVprec are shown between the jaw and finger. So we compared the relative changes from pretraining to posttraining to show the differences in the motor control of the jaw and finger after the training period. The result shows that relative changes differ significantly from session 1 to session 2 between the jaw and finger. The value of the relative change of jaw movements was more than that of the finger showing that the control of the jaw is much better than that of the finger.

The mean and standard deviation of (a) Daccu (accuracy) and (b) CVprec (precision) for different sessions (before, after, and relative changes) of different sites (jaw, finger) with or without visual feedback. ∗ indicates a significant difference ...
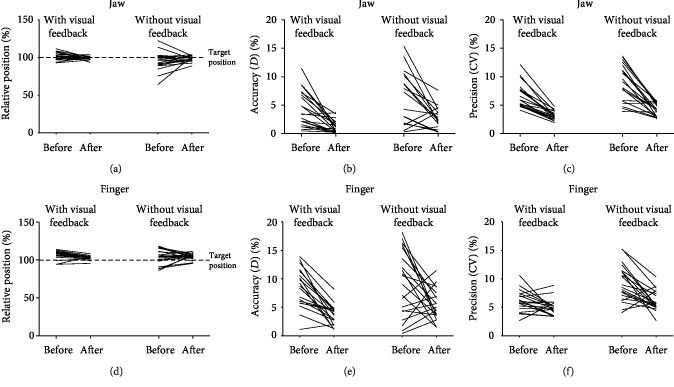
(a) Individual values of relative target position, (b) Daccu (accuracy), (c) CVprec (precision) for jaw open-close movements and (d) individual values of relative target position, (e) Daccu (accuracy), and (f) CVprec (precision) for finger lifting movements ...

Mean values and standard deviation of Daccu and CVprec and relative changes in Daccu and CVprec of 20 participants for jaw open-close and finger lifting movements with or without visual feedback before or after 3-day motor training.
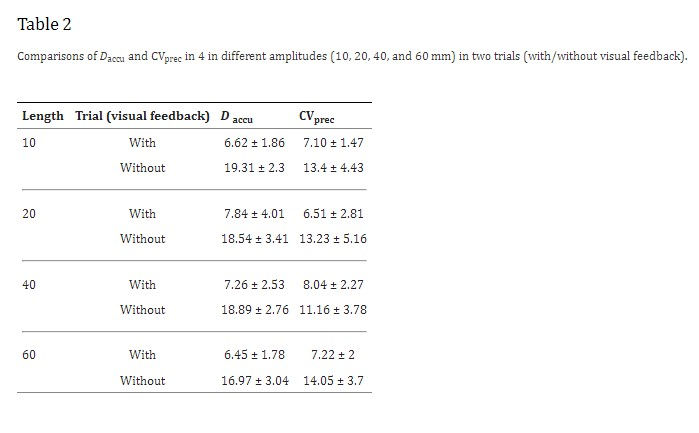
Comparisons of Daccu and CVprec in 4 in different amplitudes (10, 20, 40, and 60 mm) in two trials (with/without visual feedback).
3.1. Comparison of Male and Female
Before motor training, we found that there were significant differences in the Daccu of jaw movements with visual feedback (ANOVA, F = 6.134, df = 1, P = 0.023) between males and females. However, males and females did not differ significantly in the CVprec of jaw movement with visual feedback (ANOVA, F = 0.441, df = 1, P = 0.515) and the Daccu and CVprec of jaw movements without visual feedback (ANOVA, F = 1.191, df = 1, P = 0.289; ANOVA, F = 1.145, df = 1, P = 0.299, respectively). There were no significant differences of the Daccu and CVprec of finger movement with visual feedback (ANOVA, F = 0.611, df = 1, P = 0.444; ANOVA, F = 3.628, df = 1, P = 0.073, respectively) nor without visual feedback (ANOVA, F = 1.596, df = 1, P = 0.223; ANOVA, F = 0.114, df = 1, P = 0.739, respectively).
3.2. Daccu
There were statistically significant effects of session (ANOVA, F = 41.183, df = 1, P < 0.001), trial (ANOVA, F = 11.795, df = 1, P = 0.001) and site (ANOVA, F = 8.736, df = 1, P = 0.004) for Daccu.
In jaw open-close movements, Daccu in trial 2 (without visual feedback) was significantly higher than that in trial 1 (with visual feedback) in session 1 (pretraining) (ANOVA, F = 5.821, df = 1, P = 0.021) and session 2 (posttraining) (ANOVA, F = 8.150, df = 1, P = 0.007). Moreover, in trials 1 and 2, Daccu were higher in session 1 than in session 2 (ANOVA, F = 17.867, df = 1, P < 0.001; ANOVA, F = 9.316, df = 1, P = 0.004, respectively).
In finger lifting movements, Daccu in trial 2 was significantly higher than that in trial 1 in session 2 (ANOVA, F = 5.522, df = 1, P = 0.024) but not significantly different than that in session 1 (ANOVA, F = 0.337, df = 1, P = 0.565). Daccu in session 1 was significantly higher than that in session 2 both in trial 1 and in trial 2 (ANOVA, F = 28.613, df = 1, P < 0.001; ANOVA, F = 7.011, df = 1, P = 0.012, respectively).
The direct comparison of the finger lifting movements demonstrated significantly lower Daccu values than that of the jaw open-close movements (i.e., better accuracy) in trial 1 (ANOVA, F = 14.416, df = 1, P = 0.001) and trial 2 (ANOVA, F = 28.551, df = 1, P < 0.001) for session 1. Daccu for the jaw open-close movements was also significantly lower than that for the finger lifting movements in trial 2 (ANOVA, F = 7.511, df = 1, P = 0.009) for session 2 (Table 1). Moreover, the relative changes of Daccu from session 1 to session 2 (before-after training) in trial 2 were significantly different between jaw and finger movements (ANOVA; F = 6.211, df = 1, P = 0.017) indicating greater improvement in accuracy for the jaw movements compared to the finger movements from pre- to posttraining.
3.3. CVprec
For CVprec, there were also significant effects of session (ANOVA, F = 89.346, df = 1, P < 0.001), trial (ANOVA, F = 32.752, df = 1, P < 0.001), and site (ANOVA, F = 5.591, df = 1, P = 0.019). There were significant interactions between session and trial (ANOVA, F = 5.875, df = 1, P = 0.017), and the interaction between session and site was significant (ANOVA, F = 9.790, df = 1, P = 0.002) (Table 3).

Comparisons of Daccu and CVprec in 20 participants at two sites (jaw and finger) in two trials (with/without visual feedback) over two sessions (before/after 3-day training) by three-way factorial analysis of variance (ANOVA).
CVprec values for jaw open-close movements of trial 1 were lower than those of trial 2 in session 1 (ANOVA, F = 6.894, df = 1, P = 0.012) and session 2 (ANOVA, F = 13.114, df = 1, P = 0.001). In trials 1 and 2, CVprec were lower in session 2 than in session 1 (ANOVA, F = 50.328, df = 1, P < 0.001; ANOVA, F = 38.546, df = 1, P < 0.001, respectively).
For finger lifting movements, CVprec in trial 2 were significantly higher than those in trial 1 in session 1 (ANOVA, F = 14.572, df = 1, P < 0.001) and in session 2 (ANOVA, F = 5.166, df = 1, P = 0.029). CVprec in session 1 were significantly higher than those in session 2 in trial 1 and trial 2 (ANOVA, F = 4.805, df = 1, P = 0.035; ANOVA, F = 14.479, df = 1, P = 0.001, respectively).
CVprec values of jaw open-close movements were significantly lower in trial 1 (ANOVA, F = 26.302, df = 1, P < 0.001) and trial 2 (ANOVA, F = 16.283, df = 1, P < 0.001) for session 2 compared with those of finger lifting movements. There were no significant differences in trial 1 or trial 2 of session 2 (Table 1). The relative changes of CVprec from session 1 to session 2 (before-after training) in trial 1 and trial 2 were both significantly different between jaw and finger movements (ANOVA, F = 12.670, df = 1, P = 0.001; ANOVA, F = 5.275, df = 1, P = 0.027, respectively) showing that improvements in precision for the jaw movements were more remarkable compared to those for the finger movements from pre- to posttraining.
3.4. Control Experiment
The control experiment for the finger lifting movements at different amplitudes did not indicate any significant differences in Daccu or CVprec neither with (ANOVA, df = 3, F = 0.552, P = 0.650; ANOVA, df = 3, F = 0.828, P = 0.486, respectively) nor without (ANOVA; df = 3, F = 1.232, P = 0.311; ANOVA, df = 3, F = 0.844, P = 0.478, respectively) visual feedback (Figure 5).
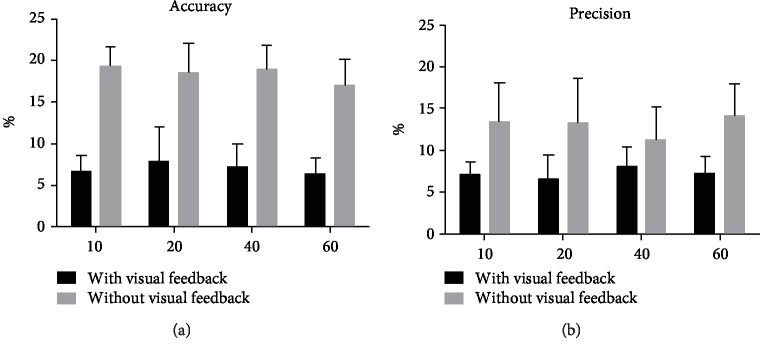
Control experiment (n = 11). The mean and standard deviation of (a) Daccu (accuracy) and (b) CVprec (precision) for different amplitudes (10, 20, 40, and 60 mm) of finger movements with or without visual feedback.
4. Discussion
The present study assessed jaw and finger motor performance during a short-term (3 days) task training and revealed a significant increase in the precision and accuracy of task performance for both the jaw and finger movements.
To promote learning of new abilities, retrieving lost skills or keeping existing skills is the purpose of neurorehabilitation but has received relatively little attention in the rehabilitation of impaired oral function, e.g., chewing and biting. A variety of factors may have influence on neurorehabilitation and effect on motor learning processes. These factors include, for example, verbal instructions and variability of training sessions, posture control, and visual feedback.
4.1. Neuroplasticity and Motor Improvement
The capacity of the nervous system to modify its organization to altered demands and environments has been termed “neuroplasticity” [21]. Neuroplasticity occurs when, for example, acquiring new skills, after damage to the nervous system and as a result of sensory deprivation. Neuroplasticity has been studied at different organizational levels of the nervous system, ranging from ion channels to synapses, neurons, neuronal columns, cortical maps, and behavior. These levels are, however, highly interlinked and interdependent. Associative learning, for example, induces changes in the release of neurotransmitters, which then may trigger a cascade of neurochemical events resulting in structural changes in the cerebral cortex such as the formation of new synapses or the reorganization of synaptic connections [22]. For instance, these structural changes could sometimes lead to an expansion of cortical maps [23]. Reorganizations of sensory cortical maps have been linked to changes in perceptual abilities measured at the behavioral level [24]. Neuroplasticity is a continuous process allowing short-term, medium-term, and long-term remodeling of the neuron-synaptic organization, with the aim of optimizing the function of neural networks during phylogenesis, ontogeny, and physiologic learning and following brain injury. Previous research has demonstrated that primary motor area (MI) neuroplasticity, as reflected in changes in intracortical microstimulation (ICMS) features or neuronal activity patterns, is associated with the learning of a novel orofacial motor task. Cortical damage, stroke, can impair orofacial sensory and motor functions in humans, which may substantiate that MI plays a strategic role not only in elemental and learned motor behaviors but also in certain aspects of chewing and swallowing, a kind of peripheral region change. The studies have utilized ICMS, reversible cold block, or single neuron recordings in MI. MI is contributing to control and regulation of mastication and swallowing which may help to explain why cortical damage, stroke, can impair orofacial sensory and motor functions in humans [25].
Motor improvement also reflects other functional changes not necessarily neuroplastic ones. Previous research in rats demonstrated that increases in forelimb motor activity can induce cortical angiogenesis which suggest that changes occur not only in neurons but also occur in glial cells and blood vessels [26, 27]. Besides, motor control training may improve the size and function of trunk muscles in elite football players which is a kind of peripheral changes [28]. The present data may however not easily be explained by such peripheral changes, and more likely, central neuroplasticity plays a more important role in the behavioral improvements observed for both the jaw and finger movements.
4.2. Effects of Visual Feedback
It is unclear whether cognition and motor control are parallel and interactive or serial and independent processes. According to one view, cognitive control refers to a set of modality-nonspecific processes that act on supramodal representations and precede response modality-specific motor processes [29]. In the present study, it was shown that observation of your own jaw and finger movements to reach a specific target position was associated with more accurate and precise movements compared to when no visual cues were provided. Interestingly, this was observed for both the jaw and finger movements; however, in more daily activities, visual inspection of your fingers is obviously feasible whereas a mirror is required to allow a visual inspection of your jaw movements. Nevertheless, both the spinal and trigeminal systems seem to perform better when under visual control. In terms of development of specific training paradigms for neurorehabilitation of lost jaw function, it seems valuable to use mirrors for individuals to obtain visual feedback.
Participants can, indeed, acquire neural representations on the basis of visual information [30]. Further, while motor learning by observation does not depend on conscious awareness of the individuals, the tendency for an unrelated movement task to significantly reduce the ability of participants to learn by observing indicates that the implicit engagement of motor systems is required [17].
It has been demonstrated that the force control of jaw muscles could be improved when the isometric contractions of muscles were guided with visual feedback [31, 32]. In a previous study, there were indications that observation of a movement activates cortical motor areas involved in producing the same movement [33]. This suggests that motor systems are involved in acquiring neural representations during observation, which also will have occurred in the present study for both the jaw- and finger-movement tasks.
4.3. Effects of Training
Motor adaptation of the jaw motor system has been studied by many investigators [34, 35]. It has been suggested that it would be used in the future with a great development prospect to get adaptation during a long-term training by undergoing force-controlled balancing tasks [35]. In the study reported by Kumar et al. [14], increased precision and shorter duration of the tasks were observed following a short-term training task (about 30 min), which may suggest a better fine oral motor control and optimization of jaw movements. A study using transcranial magnetic stimulation (TMS) to probe cortical excitability demonstrated that repeated and standardized tooth-clenching tasks triggered significant neuroplastic changes in the corticomotor control of the jaw-closing muscles [5].
In the present study, significant differences were found for both jaw and finger movements between pre- and posttraining. Participants executed the repeated movements more accurately and precisely after they underwent a 3-day motor training program. It has been demonstrated that motor learning is highly related to motor cortex plasticity [36], and a previous study suggested that neuroplastic change occurs in the corticomotor pathways within 30 min after a repetitive tongue training task [4].
It seems reasonable to suggest that practice of repeated movements or tasks is essential for learning and acquisition of new motor skills [37]. Indeed, skilled motor training will include the optimization of different action phases [38] and also lead to cortical reorganization and adaptation of the behavior of single motor units [8, 39]. Acquisition of skilled movement normally refers to processes by which movements performed through repeated practice will be executed more effortlessly and it may also be associated with significant reorganization of the motor cortex [40]. In this study, only vertical jaw and finger movements were recorded, and further studies will be needed to directly demonstrate any neuroplastic changes underlying the improved performance for both the jaw and finger, e.g., using TMS or fMRI studies.
4.4. Differences between Jaw and Finger Movements
It was observed that both the Daccu (accuracy) and CVprec values (precision) were significantly different between jaw and finger movements. Indeed, the differences for the changes of Daccu and CVprec were significantly different from session 1 to session 2 between the jaw and finger and demonstrated that the accuracy and precision for jaw open-close movements were improved more than those for finger lifting movements after participants had trained for 3 days. There are several distinctive properties of the jaw muscles and their control that distinguish them from the spinally innervated neck, trunk, and limb muscles. In terms of movement and sensorimotor control, orofacial muscles contract bilaterally and are under bilateral sensorimotor control; limb muscles contract unilaterally or alternating left/right contraction and are under unilateral sensorimotor control. According to the location of the motor neuron cell bodies, orofacial muscles are controlled from distinctive brainstem motor nuclei, and limb muscles are controlled from the ventral horn of the spinal cord. Besides, with regard to muscle spindles and some periodontal mechanoreceptors, orofacial muscles are controlled from neurons within the CNS (in the mesencephalic nucleus), and limb muscles are controlled outside the CNS [41]. Previous studies also showed that a distinct difference was observed in the mechanisms of motor control in the trigeminally innervated jaw muscles compared to those in spinally innervated finger muscles when similar motor tasks were performed [15, 42]. It has been suggested that this may lead to differences in motor patterns and control mechanisms between spinally innervated and trigeminally innervated muscle contractions. In the study comparing the cortical activity of bilateral light fist clenching and light tooth clenching in healthy individuals [16], there were indications that the tooth clenching task was more complex than the hand motor task in terms of the associated cortical activation patterns [16]. It was suggested that the jaw motor system may be a more complex system than the finger. The present findings may be interpreted in the same manner namely that improvements of performance are more likely to occur in the jaw motor system after short-term training than the hand motor system.
However, the recent findings from a study of Kumar et al. [15] reported that force control within the jaw motor system was more variable than the fingers indicating disassociation between accuracy and precision due to repeated performance of an oral motor task and digital motor task. It was proposed that there may be mechanistic differences between force and motor control as such.
4.5. Methodological Limitations
Many factors may have effects on motor learning including age, anatomical characteristics, specifics of the training sessions, the individual's active participation and motivation, positive and negative learning transfer, posture control, and memory. An element of a test-retest measurement incorporated in the outcome needs to be considered for this kind of training studies. All these factors may be clinically relevant and may provide the basis for emerging or new lines of research having to do with retraining of sensorimotor function in neurological patients and in patients undergoing oral rehabilitation [25, 43]. In this study, only one direction of movement was trained and tested, and the sample size was relatively small with only young and healthy participants being included. Another limitation in the present study could be the fixed amplitude (20 mm) for the finger movement when the amplitude for the jaw movement was set to 50% of the individual maximal jaw opening. Although no differences in accuracy and precision were found between different ranges of finger lift movements, including 50% of the maximal finger lift capacity in the control experiment in this study, one could argue that the jaw and finger movements were still quite different making a direct comparison difficult. However, in terms of the absolute jaw opening distance, 50% corresponds approximately to 20-25 mm [44–46]. In combination with the fact that accuracy and precision did not change over a range of different finger lift movements, it seems that the present findings are valid and, indeed, suggest a remarkable difference in training-evoked effects between the spinal and trigeminal motor systems.
Another issue to be discussed in this study is related to the participant's memory of the different movements. According to Ebbinghaus' classical forgetting curve, memory retention reduced from 100% to 58.2% only after 20 min after the first learning task [47]. Memory mechanisms may also play an important role in motor learning and training. However, in the present study, all series of movements were performed in a few minutes after the initial learning so that further studies would be needed to demonstrate a clear decreasing or increasing trend in performance in the consecutive movements at different time points. It should be mentioned that the outcome parameters in the present study also may include the natural variability of the task performance; however, this is inherent in the study of training-related effects due to the repeated task performance. In addition, the fingers work very often under a visual control, while the jaw works almost always without visual feedback. The influence of visual feedback was different between spinally innervated and trigeminally innervated muscles, and the specific mechanism of force execution from muscle activity seems to be different between the jaw and hand motor systems, which may affect the results of the experiment; so further follow-up research could explore potential mechanisms related to the force control of hand and jaw muscles in the peripheral nervous system [17]. Finally, further studies would also be needed to examine the effect of motor cortex neuroplasticity related to longer training paradigms and longer follow-up periods and in larger sample sizes and with more complex types of movement training.
5. Conclusion
In conclusion, the accuracy and precision of standardized jaw and finger movements are dependent on visual feedback and appear to improve more by training in the trigeminal system. Somatosensory plus visual feedback, in addition to proprioception, provides a better sense of body (jaw and finger) position before a movement is being carried-out; sensory inputs and subsequent sensorimotor integration allow for corrections in movements while they occur; if repeated enough times, at enough intensity, these may generate plastic changes, such as generation of new networks, reorganization of motor representations, and changes in neuron excitability which will lead to changes in motor programs so that future movements are performed more accurately and precisely. Each of the above changes in motor functions involves different plastic changes in the brain occurring at different points of time.
Acknowledgments
The study was supported by the National Natural Science Foundation of China (81830031 and 81570959), State Key Lab of Reproductive Medicine of Nanjing Medical University (JX116GSP20171416), the Priority Academic Program Development of Jiangsu Higher Education Institutions (PAPD-2018-87), the Natural Science Foundation of Jiangsu Province (BL2014073 and 15KJA320002 to Lin Wang), the Research Topics of Cadre Health Care in Jiangsu Province, China (No. BJ116030), and the Jiangsu Provincial Key Medical Discipline (zdxka2016026). This study was also supported by the Orofacial Pain & TMD Research Unit, Institute of Stomatology, Affiliated Hospital of Stomatology, Nanjing Medical University, Nanjing, P. R. China.
Data Availability
Raw data were generated using a kinesiograph (K7/CMS; Myotronics, Inc., USA) and commercial software program (K7 Program, Myotronics, Inc., USA). Derived data supporting the findings of this study are available from the corresponding authors upon request.
Disclosure
Y.C. and S.W. are regarded as co-first author.
Conflicts of Interest
No conflicts of interest, financial or otherwise, are declared by the authors.
Authors' Contributions
Y.C. and S.W. were responsible for conducting the study, data collection and management, and data analysis and drafted the manuscript. L.Y. prepared Figures Figures1,1, ,22 and and4.4. J.Z., K.W., and P.S. participated in the design of the study and revised the manuscript. P.S. conceived the idea behind the study. All authors read and approved the final manuscript. Y.C. and S.W. contributed equally to this work and are regarded as co-first authors. Yinan Chen and Song Wu contributed equally.
Article information
Neural Plast. 2019; 2019: 9593464.
Published online 2019 Nov 18. doi: 10.1155/2019/9593464
PMCID: PMC6885803
PMID: 31827500
Yinan Chen, 1 , 2 , 3 Song Wu, 1 , 2 Zhengting Tang, 1 , 2 Jinglu Zhang, 1 , 2 Lin Wang, 2 Linfeng Yu, 1 Kelun Wang, 1 , 4 and Peter Svensson 5 , 6 , 7
1Orofacial Pain & TMD Research Unit, Institute of Stomatology, Department of Polyclinic, Affiliated Hospital of Stomatology, Nanjing Medical University, Nanjing, China
2Jiangsu Key Laboratory of Oral Diseases, Nanjing Medical University; Department of Polyclinic, Affiliated Hospital of Stomatology, Nanjing Medical University, Nanjing, China
3Department of Dentistry, BenQ Medical Center, The Affiliated BenQ Hospital of Nanjing Medical University, Nanjing, Jiangsu Province, China
4Center for Sensory-Motor Interaction (SMI), Aalborg University, Aalborg, Denmark
5Section of Orofacial Pain and Jaw Function, Department of Dentistry and Oral Health, Aarhus University, Denmark
6Department of Dental Medicine, Karolinska Institutet, Huddinge, Sweden
7Scandinavian Center for Orofacial Neurosciences (SCON), Denmark
Corresponding author.
Jinglu Zhang: nc.ude.umjn@mylljhz; Lin Wang: nc.ude.umjn@306wl
Academic Editor: Gabriela Delevati Colpo
Received 2019 May 30; Revised 2019 Sep 1; Accepted 2019 Sep 24.
Copyright © 2019 Yinan Chen et al.
This is an open access article distributed under the Creative Commons Attribution License, which permits unrestricted use, distribution, and reproduction in any medium, provided the original work is properly cited.
This article has been corrected. See Neural Plast. 2021; 2021: 9760392.
Articles from Neural Plasticity are provided here courtesy of Hindawi Limited
References
1. Johnston M. V. Clinical disorders of brain plasticity. Brain Development. 2004;26(2):73–80. doi: 10.1016/S0387-7604(03)00102-5. [PubMed] [CrossRef] [Google Scholar]
2. Boudreau S., Romaniello A., Wang K., Svensson P., Sessle B. J., Arendt-Nielsen L. The effects of intra-oral pain on motor cortex neuroplasticity associated with short-term novel tongue-protrusion training in humans. Pain. 2007;132(1):169–178. doi: 10.1016/j.pain.2007.07.019. [PubMed] [CrossRef] [Google Scholar]
3. Martin L., Christoph B., Niels B., Anders S., Cohen L. G. Motor learning elicited by voluntary drive. Brain. 2003;126(4):866–872. doi: 10.1093/brain/awg079. [PubMed] [CrossRef] [Google Scholar]
4. Svensson P., Romaniello A., Arendt-Nielsen L., Sessle B. J. Plasticity in corticomotor control of the human tongue musculature induced by tongue-task training. Experimental Brain Research. 2003;152(1):42–51. doi: 10.1007/s00221-003-1517-2. [PubMed] [CrossRef] [Google Scholar]
5. Iida T., Komiyama O., Obara R., Baad-Hansen L., Kawara M., Svensson P. Repeated clenching causes plasticity in corticomotor control of jaw muscles. European Journal of Oral Sciences. 2014;122(1):42–48. doi: 10.1111/eos.12101. [PubMed] [CrossRef] [Google Scholar]
6. Iida T., Overgaard A., Komiyama O., et al. Analysis of brain and muscle activity during low‐level tooth clenching – a feasibility study with a novel biting device. Journal of Oral Rehabilitation. 2014;41(2):93–100. doi: 10.1111/joor.12128. [PubMed] [CrossRef] [Google Scholar]
7. Garry M. I., Kamen G., Nordstrom M. A. Hemispheric differences in the relationship between corticomotor excitability changes following a fine-motor task and motor learning. Journal of Neurophysiology. 2004;91(4):1570–1578. doi: 10.1152/jn.00595.2003. [PubMed] [CrossRef] [Google Scholar]
8. Muellbacher W., Ziemann U., Boroojerdi B., Cohen L., Hallett M. Role of the human motor cortex in rapid motor learning. Experimental Brain Research. 2001;136(4):431–438. doi: 10.1007/s002210000614. [PubMed] [CrossRef] [Google Scholar]
9. Perez M. A., Lungholt B. K., Nyborg K., Nielsen J. B. Motor skill training induces changes in the excitability of the leg cortical area in healthy humans. Experimental Brain Research. 2004;159(2):197–205. doi: 10.1007/s00221-004-1947-5. [PubMed] [CrossRef] [Google Scholar]
10. Lund J. P. Mastication and its control by the brain stem. Critical Reviews in Oral Biology & Medicine. 1991;2(1):33–64. doi: 10.1177/10454411910020010401. [PubMed] [CrossRef] [Google Scholar]
11. Dellow P. G., Lund J. P. Evidence for central timing of rhythmical mastication. Journal of Physiology. 1971;215(1):1–13. doi: 10.1113/jphysiol.1971.sp009454. [PMC free article] [PubMed] [CrossRef] [Google Scholar]
12. Lund J. P. Chew before you swallow. Progress in Brain Research. 2011;188:219–228. doi: 10.1016/B978-0-444-53825-3.00020-6. [PubMed] [CrossRef] [Google Scholar]
13. Sessle B. J., Adachi K., Avivi-Arber L., et al. Neuroplasticity of face primary motor cortex control of orofacial movements. Archives of Oral Biology. 2007;52(4):334–337. doi: 10.1016/j.archoralbio.2006.11.002. [PubMed] [CrossRef] [Google Scholar]
14. Kumar A., Grigoriadis J., Trulsson M., Svensson P., Svensson K. G. Effects of short-term training on behavioral learning and skill acquisition during intraoral fine motor task. Neuroscience. 2015;306:10–17. doi: 10.1016/j.neuroscience.2015.06.065. [PubMed] [CrossRef] [Google Scholar]
15. Kumar A., Tanaka Y., Grigoriadis A., Grigoriadis J., Trulsson M., Svensson P. Training-induced dynamics of accuracy and precision in human motor control. Scientific Reports. 2017;7(1):p. 6784. doi: 10.1038/s41598-017-07078-y. [PMC free article] [PubMed] [CrossRef] [Google Scholar]
16. Iida T., Kato M., Komiyama O., et al. Comparison of cerebral activity during teeth clenching and fist clenching: a functional magnetic resonance imaging study. European Journal of Oral Sciences. 2010;118(6):635–641. doi: 10.1111/j.1600-0722.2010.00784.x. [PubMed] [CrossRef] [Google Scholar]
17. Iida T., Komiyama O., Obara R., Baad-Hansen L., Kawara M., Svensson P. Influence of visual feedback on force-EMG curves from spinally innervated versus trigeminally innervated muscles. Archives of Oral Biology. 2013;58(3):331–339. doi: 10.1016/j.archoralbio.2012.12.005. [PubMed] [CrossRef] [Google Scholar]
18. Mcauley J. D., Miller N. S. Picking up the pace: effects of global temporal context on sensitivity to the tempo of auditory sequences. Perception & Psychophysics. 2007;69(5):709–718. doi: 10.3758/BF03193773. [PubMed] [CrossRef] [Google Scholar]
19. Merchant H., Zarco W., Prado L. Do we have a common mechanism for measuring time in the hundreds of millisecond range? Evidence from multiple-interval timing tasks. Journal of Neurophysiology. 2008;99(2):939–949. doi: 10.1152/jn.01225.2007. [PubMed] [CrossRef] [Google Scholar]
20. Leles C. R., Compagnoni M. A., Souza R. F. ., Barbosa D. B. Kinesiographic study of mandibular movements during functional adaptation to complete dentures. Journal of Applied Oral Science. 2003;11(4):311–318. doi: 10.1590/s1678-77572003000400007. [PubMed] [CrossRef] [Google Scholar]
21. Bavelier D., Neville H. J. Cross-modal plasticity: where and how? Nature Reviews Neuroscience. 2003;3(6):443–452. doi: 10.1038/nrn848. [PubMed] [CrossRef] [Google Scholar]
22. Rosenzweig M. R., Leiman A. L., Breedlove S. M. Biological Psychology. Sunderland, MA: Sinauer Associates; 2002. Emotions and Mental Disorders. [Google Scholar]
23. Weinberger N. M. Specific long-term memory traces in primary auditory cortex. Nature Reviews Neuroscience. 2004;5(4):279–290. doi: 10.1038/nrn1366. [PMC free article] [PubMed] [CrossRef] [Google Scholar]
24. Recanzone G. H., Schreiner C. E., Merzenich M. M. Plasticity in the frequency representation of primary auditory cortex following discrimination training in adult owl monkeys. Journal of Neuroscience. 1993;13(1):87–103. [PMC free article] [PubMed] [Google Scholar]
25. Avivi-Arber L., Martin R., Lee J. C. Face sensorimotor cortex and its neuroplasticity related to orofacial sensorimotor functions. Archives of Oral Biology. 2011;56(12):1440–1465. doi: 10.1016/j.archoralbio.2011.04.005. [PubMed] [CrossRef] [Google Scholar]
26. Takahashi K., Avivi-Arber L. Society for neuroscience 2017 satellite symposium on feeding and swallowing: from neural mechanisms to rehabilitation. Dysphagia. 2018;33(2):268–271. doi: 10.1007/s00455-018-9887-6. [PubMed] [CrossRef] [Google Scholar]
27. Kleim J. A., Markham J. A., Vij K., Freese J. L., Ballard D. H., Greenough W. T. Motor learning induces astrocytic hypertrophy in the cerebellar cortex. Behavioural Brain Research. 2007;178(2):244–249. doi: 10.1016/j.bbr.2006.12.022. [PMC free article] [PubMed] [CrossRef] [Google Scholar]
28. Mendis M. D., Hides J. A. Effect of motor control training on hip muscles in elite football players with and without low back pain. Journal of Science and Medicine in Sport. 2016;19(11):866–871. doi: 10.1016/j.jsams.2016.02.008. [PubMed] [CrossRef] [Google Scholar]
29. Rietbergen M., Roelofs A., den Ouden H. Disentangling cognitive from motor control: influence of response modality on updating, inhibiting, and shifting. Acta Psychologica. 2018;191:124–130. doi: 10.1016/j.actpsy.2018.09.008. [PubMed] [CrossRef] [Google Scholar]
30. Ramachandran V. S., Altschuler E. L. The use of visual feedback, in particular mirror visual feedback, in restoring brain function. Brain. 2009;132(7):1693–1710. doi: 10.1093/brain/awp135. [PubMed] [CrossRef] [Google Scholar]
31. Lewis G. R., Yemm R. Measurement of the ability to control jaw muscle forces in man. Journal of Dental Research. 1983;62(7):821–824. doi: 10.1177/00220345830620071201. [PubMed] [CrossRef] [Google Scholar]
32. Testa M., Rolando M., Roatta S. Control of jaw-clenching forces in dentate subjects. Journal of Orofacial Pain. 2011;25(3):250–260. [PubMed] [Google Scholar]
33. Rizzolatti G., Fogassi L., Gallese V. Neurophysiological mechanisms underlying the understanding and imitation of action. Nature Reviews Neuroscience. 2001;2(9):661–670. doi: 10.1038/35090060. [PubMed] [CrossRef] [Google Scholar]
34. Boudreau S. A., Hennings K., Svensson P., Sessle B. J., Arendt-Nielsen L. The effects of training time, sensory loss and pain on human motor learning. Journal of Oral Rehabilitation. 2010;37(9):704–718. doi: 10.1111/j.1365-2842.2010.02103.x. [PubMed] [CrossRef] [Google Scholar]
35. Hellmann D., Giannakopoulos N. N., Blaser R., Eberhard L., Rues S., Schindler H. J. Long‐term training effects on masticatory muscles. Journal of Oral Rehabilitation. 2011;38(12):912–920. doi: 10.1111/j.1365-2842.2011.02227.x. [PubMed] [CrossRef] [Google Scholar]
36. Hosp J. A., Luft A. R. Cortical plasticity during motor learning and recovery after ischemic stroke. Neural Plasticity. 2011;2011:9. doi: 10.1155/2011/871296.871296 [PMC free article] [PubMed] [CrossRef] [Google Scholar]
37. Lee T. D., Swanson L. R., Hall A. L. What is repeated in a repetition? Effects of practice conditions on motor skill acquisition. Physical Therapy. 1991;71(2):150–156. doi: 10.1093/ptj/71.2.150. [PubMed] [CrossRef] [Google Scholar]
38. Säfström D., Flanagan J. R., Johansson R. S. Skill learning involves optimizing the linking of action phases. Journal of Neurophysiology. 2013;110(6):1291–1300. doi: 10.1152/jn.00019.2013. [PubMed] [CrossRef] [Google Scholar]
39. Duchateau J., Semmler J. G., Enoka R. M. Training adaptations in the behavior of human motor units. Journal of Applied Physiology. 2007;101(6):1766–1775. doi: 10.1152/japplphysiol.00543.2006. [PubMed] [CrossRef] [Google Scholar]
40. Pascualleone A., Grafman J., Hallett M. Modulation of cortical motor output maps during development of implicit and explicit knowledge. Science. 1994;263(5151):1287–1289. doi: 10.1126/science.8122113. [PubMed] [CrossRef] [Google Scholar]
41. Avivi-Arber L., Sessle B. J. Jaw sensorimotor control in healthy adults and effects of ageing. Journal of Oral Rehabilitation. 2018;45(1):50–80. doi: 10.1111/joor.12554. [PubMed] [CrossRef] [Google Scholar]
42. Zhang H., Kumar A., Luo X., Svensson K., Trulsson M., Svensson P. Effect of short-term training on fine motor control in trigeminally innervated versus spinally innervated muscles. Human Movement Science. 2018;58:132–139. doi: 10.1016/j.humov.2018.01.013. [PubMed] [CrossRef] [Google Scholar]
43. Roatta S., Rolando M., Notaro V., Testa M., Bassi F., Passatore M. Objective assessment of mandibular motor control using a ‘reach‐and‐hold’ task. Journal of Oral Rehabilitation. 2011;38(10):737–745. doi: 10.1111/j.1365-2842.2011.02215.x. [PubMed] [CrossRef] [Google Scholar]
44. Mezitis M., Rallis G., Zachariades N. The normal range of mouth opening. Journal of Oral & Maxillofacial Surgery. 1989;47(10):1028–1029. doi: 10.1016/0278-2391(89)90174-2. [PubMed] [CrossRef] [Google Scholar]
45. Li X. Y., Jia C., Zhang Z. C. The normal range of maximum mouth opening and its correlation with height or weight in the young adult Chinese population. Journal of Dental Sciences. 2017;12(1):56–59. doi: 10.1016/j.jds.2016.09.002. [PMC free article] [PubMed] [CrossRef] [Google Scholar]
46. Gallagher C., Gallagher V., Whelton H., Cronin M. The normal range of mouth opening in an Irish population. Journal of oral Rehabilitation. 2004;31(2):110–116. doi: 10.1046/j.0305-182x.2003.01209.x. [PubMed] [CrossRef] [Google Scholar]
47. Murre J. M., Dros J. Replication and analysis of Ebbinghaus’ forgetting curve. PLoS One. 2015;10(7, article e0120644) doi: 10.1371/journal.pone.0120644. [PMC free article] [PubMed] [CrossRef] [Google Scholar]
Citation
Comments